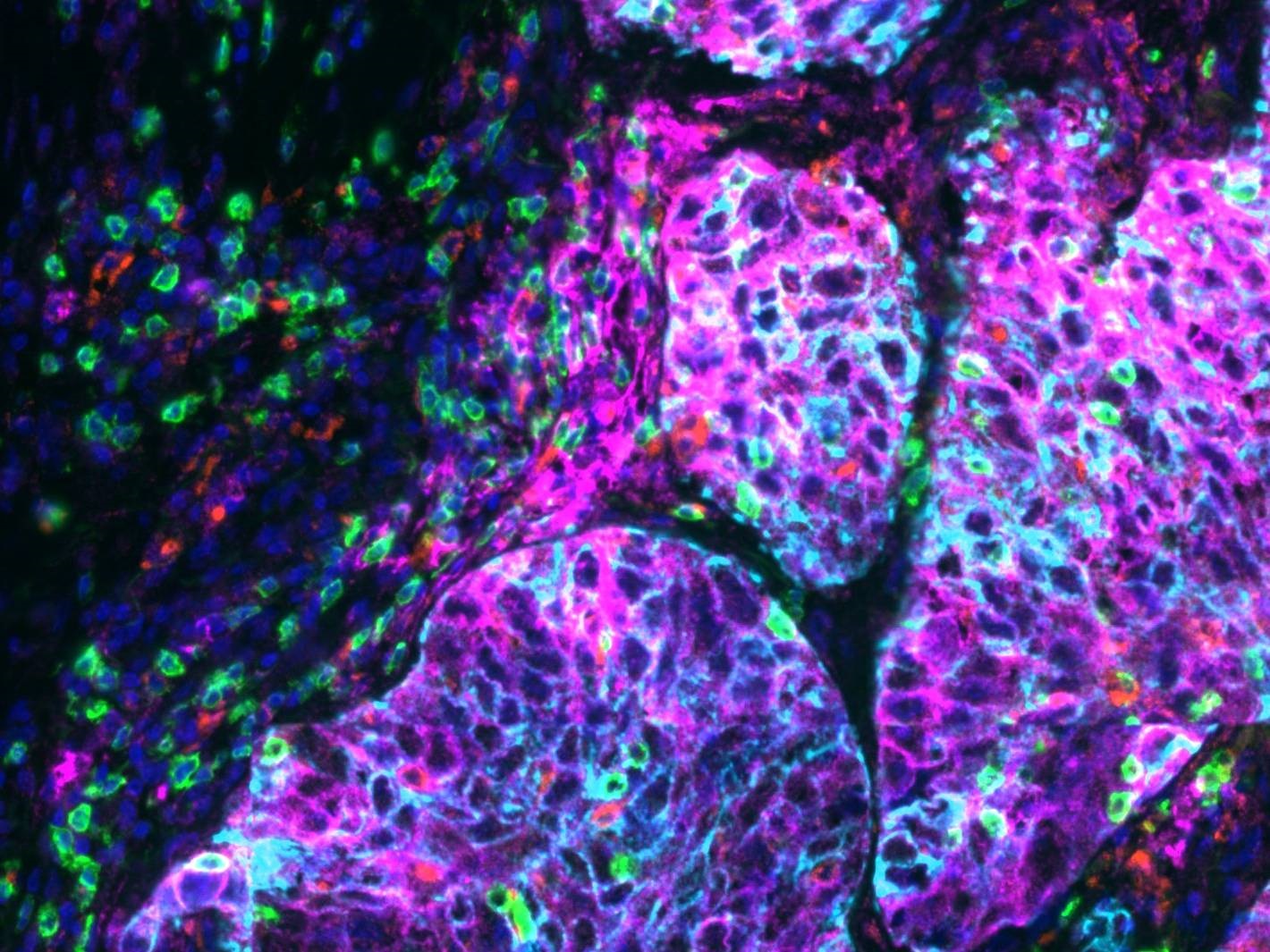
Figure 1: Lung tissue imaged on a SLIDEVIEW™ VS200 at 20X, stained with an Ultivue PD-L1 kit multiplex kit; Dapi: Nuclear Counterstain, FITC: CD8, TRITC: CD68, Cy5: PD-L1, Cy7: panCK. Image data courtesy of Ultivue, Inc.
by David Bryce, Applications Specialist, Life Science, Evident
Whole slide scanning has emerged as an invaluable tool in a range of research and clinical applications in recent years. Given the large areas that must be covered, typical approaches involve scanning slides at low magnification (10x–20x) and have been limited in application to the detection and analysis of objects resolvable at these magnifications.
Recently, numerous improvements have been made that make high-resolution slide scanning possible. However, several tradeoffs exist when considering low- versus high-resolution scanning. In comparison, low-resolution imaging—here defined as images collected at 2x–20x magnification—provides high acquisition rates (especially for large area samples) and a macro-scale overview of features or structures, while keeping file sizes small and data volumes manageable.
Many applications, however, require much more detailed images. For applications where precise localization is needed, such as those that rely on multiplexing for colocalization of multiple biomarkers, scanning at low magnification cannot supply adequate resolution for extracting meaningful information. For these applications, higher resolution images must be collected, demanding scanning at magnifications greater than 20 times. Multiplexing techniques apply fluorescent probes to a variety of biomarkers in a sample, either sequentially or simultaneously, in order to understand biomarker interactions in situ (see Figure 1). Whole slide imaging of these multiplexed systems aims to understand the spatial distribution of biomarkers within individual cells as well as observe distributions throughout the tissue microenvironment of the sample (such as within a cancer tumor). Protein distribution within individual cells is known to change in response to stimuli, such as changes in environment, disease response, or drug treatment, and these responses can fluctuate from cell to cell in a given population.
Increasing resolution of whole slide scanning enables the resolution of proteins within subcellular structures (i.e., within mitochondria), offering researchers unique insights into cellular processes in response to applied stimuli. Such subcellular structures can be approximately 0.5 microns in size, so gaining meaningful information about protein localization within such structures requires resolution much better than can be provided by a standard 20x air objective. Adapting whole slide imaging to provide high-resolution images provides a powerful tool that can offer insight into both inter- and intra-cellular behavior. Collecting high-resolution images capable of providing this information typically requires longer scanning times producing larger digital image files, and it can require oil immersion objectives and other specialized hardware—all presenting challenges to throughput. When selecting a slide scanning microscope, it is important to understand these tradeoffs and practical solutions, as well as the resolution needs of the questions one hopes to address.
Challenges of Achieving High-Throughput, High-Resolution Whole Slide Scanning
Several trials must be overcome for high-resolution whole slide imaging to be a workable tool for researchers. Many whole slide imaging systems use a single fixed objective lens for imaging. To offer imaging at higher resolution in these systems, a magnifying lens can be introduced into the light path, offering scanning capabilities at higher magnification when desired (i.e., a 2x lens to increase the magnification from 20x to 40x). Alternatively, increases in effective magnification can be achieved by adjusting pixel binning of the camera used in image acquisition. While these methods can offer higher magnification and may improve the resolution of scanned images to some degree, the optical resolution of the system may still be limited by the numerical aperture (NA) of the fixed objective lens.
Numerical aperture is important because it indicates the resolving power of a lens. The size of the finest detail that can be resolved (the resolution) is described by the Abbe diffraction limit:
Where d represents the minimum resolvable distance between two objects, λ is the wavelength of the light, and NA is the numerical aperture of the chosen lens.
A lens with a larger numerical aperture will be able to visualize finer details than a lens with a smaller numerical aperture. For example, a 20x air objective lens with a NA of 0.8 provides resolution of about 250 nanometers when collecting 400 nm light, while a 40x oil immersion lens with a NA of 1.4 improves that resolution to approximately 140 nanometers—an improvement in resolution of 1.8 times. This improvement in resolution enables the system to resolve protein localization within subcellular structures (such as within individual mitochondria). Assuming they are quality diffraction-limited optics, lenses with larger numerical apertures also collect more light and will provide a brighter image but with a shallower depth of field. For practical purposes, the minimum separation distance described above is sometimes rearranged to describe the useful range of magnification for a microscope system (objective lens, eyepieces, camera adapter, etc.). This range is defined as 500–1000 times the NA of the objective lens. These bounds of useful magnification may be beneficial when considering capabilities of systems with fixed objective lenses, where multiple magnifying elements or camera pixel binning may be used to increase effective magnification for high-resolution scanning.
Moving to higher magnification objective lenses typically provides higher NA but can require the addition of immersion media to slides before scanning, either manually or using specialized hardware. As magnification increases, so does the number of images needed to cover a given sample area, dramatically increasing scanning times for whole image acquisition. Beyond the simple increase in time for sample acquisition, an increase in total number of scanned images dramatically increases the file size of collected and stitched whole slide images. This increase in file size requires larger storage volumes, presenting challenges for high-throughput scanning where hundreds of slides may be scanned daily, each producing gigabyte or larger image files. These large files also present computational challenges for rendering and processing collected images. Additionally, applications that demand high-resolution scanning may require more dimensionality of data, which entails scanning at multiple Z positions to capture three-dimensional data. This further amplifies the issues of scan time, file size, and processing complexity.
Solutions to Slide Scanning Resolution and Efficiency Limitations
Figure 2: Blood sample scanned at (top) 40x magnification and (bottom) at 20x magnification zoomed to 40x.
There are several hardware improvements that can be made to counter challenges to high-resolution whole slide imaging. Implementation of high numerical aperture, high magnification objectives into slide scanning systems helps to address the fundamental resolution limits of the optical systems. Moving from a system that relies on combining a 20x objective lens with a 2x magnifying lens to a dedicated 40x objective represents an increase in optical resolution of approximately 1.2 times. Increasing the magnification of these fixed objective systems is possible; however, the fundamental resolving power of the system is limited by the NA of the objective lens, leading to empty magnification (see Figure 2). In empty magnification, the image is further enlarged but the details in the sample are not resolved any better. To improve resolution further, the NA of the objective lens used for imaging must be increased, such as by adding oil immersion objectives to the system. The improvement in optical resolution for this case increases to a factor of approximately 1.75 times, and the addition of automated immersion media delivery hardware enables uninterrupted scanning and removes the need for manual oil application. This alone does not alleviate the long timescale for scanning at high magnification, but acquisition times can be improved by optimizing several elements of the system.
Careful selection of a high-sensitivity scientific CMOS camera, as well as improvements to the efficiency of the optical pathway used, and use of objective lenses with high NA capabilities can all be combined to improve imaging rate. Even modest improvements in the light gathering efficiency of the optical system or the sensitivity of the camera used in acquisition may offer dramatic improvements in scanning time. The impacts are exponential since a single slide area may require hundreds or thousands of individual images to be acquired, particularly in the case of fluorescence images, where multiple fluorophores need to be imaged, across many fields of view, and potentially at multiple Z positions.
While scanning with these high-magnification, high-resolution objectives provides scanned images with improved resolution that may supply critical information, the size of the files generated increases dramatically. While the costs of digital storage space are increasingly more affordable, systems scanning a high volume of slides produce data at a rate that requires server-based storage solutions. Using a dedicated server for archiving scanned images supplies a two-fold benefit. First, storage volumes can be much higher, mitigating the concern of filling storage volumes during automated scanning, and second, it enables easy access to scanned data remotely. Remote access to scanned image data permits processing by a dedicated analysis system. Development of analysis software optimized for processing with powerful graphics processing units (GPUs) can accelerate processing of collected images.
Improving the Precision of Sample Detection with AI
High-resolution scanning time can be further reduced by limiting high-resolution scanning to specific regions of interest. This technique involves quickly acquiring a lower-magnification overview image and then locating regions or features of interest and targeting them for high-resolution scanning. Scanning lower-resolution overview images and finding specific areas for high-resolution scanning preserves the macroscale spatial information benefit of whole slide scanning and supplies high-resolution details where necessary. Choosing hardware that reliably localizes scans helps to mitigate both time and data volume challenges faced by scanning at high magnification. Additionally, powerful machine learning algorithms have been developed that enable researchers to train neural networks for sample detection. By automating this process, these tools can further reduce the time demands of manually finding regions of interest for high-magnification scanning. Artificial intelligence (AI) powered software also limits scanning to precisely defined regions of interest, resulting in reductions in over-scan area that can dramatically reduce scan time when one considers the reduced number of images collected over a whole tissue section with dispersed regions of interest.
Conclusion
Whole slide imaging offers a powerful tool for researchers, capable of supplying macroscale structural information as well as subcellular information. Recently, several advancements have made high-resolution scanning an attractive option for systems where scanning at 10x or 20x magnification does not meet resolution needs. High-resolution scanning presents several challenges—a long acquisition timescale that may limit throughput as well as large data volumes—when compared to lower-magnification scanning. Strategies exist to address these challenges, including carefully choosing hardware (optical components, cameras, etc.), software, and data storage modality.
About the Author: David Bryce received his PhD in Analytical Chemistry from the University of Utah in 2018, where he applied Raman and fluorescence microscopy to study molecular interactions at liquid-solid interfaces. David joined Evident in 2019 as a service engineer, supporting confocal, spinning disk, and slide scanning microscope products. David joined the life sciences applications group in 2022 to help support a range of products including the SLIDEVIEW™ VS200 slide scanner.