Featured Article
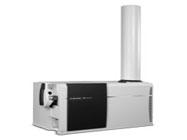
Please check out our Mass Spectrometers section for more information or to find manufacturers that sell these products.
Originally employed as an analytical tool in the basic sciences of chemistry and physics, mass spectrometry has established its utility in the clinical setting for more than a quarter of a century.1 No longer confined to reference laboratories or university-based medical centers, user-friendly, affordable, and versatile mass spectrometers show promise in multiple clinical applications, including analysis and identification of amino acids, fatty and organic acids, steroids, carbohydrates, peptides, proteins, oligonucleotides, and DNA. This article will cover the basic elements of mass spectrometry, compare existing forms of MS, and discuss the feasibility of point-of-care mass spectrometry in the future of personalized medicine.
Mass spectrometry measures the mass-to-charge ratio (m/z) of molecular and atomic species. Measuring molecular or atomic weight of a molecule or atom provides a unique “fingerprint” intrinsic to the sample.1 Ion currents produced during mass spectrometry are proportional to the amount of sample introduced, which makes possible the quantification of target analytes.
Generally, quantification can be divided into two classes: label-based (i.e., iTRAQ, cICAT, or SILAC) or label-free techniques.2 The label-free approach to quantitative mass spectrometry relies on a linear relationship between concentration of analyte and a) spectra-based measurement of signal intensity or b) spectral counting.3 Quantization strategies are central to identifying biomarkers indicative of disease.
Ionization
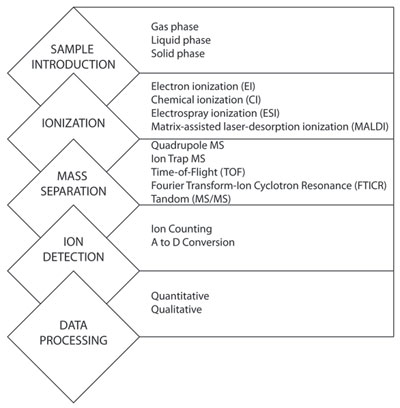
Figure 1 - Elements of mass spectrometry. The figure illustrates the conserved elements of mass spectrometry (left) and examples of different methods for each element (right).
Though multiple types and designs of mass spectrometers are commercially available (Table 1), conserved features and functions exist across all platforms (Figure 1). All mass spectrometers must convert the test sample to a charged gas. Depending on the native state of the sample, different ionization techniques can be used. Samples in gas or liquid phase can be ionized by gas chromatography or liquid chromatography, respectively. Samples introduced in a solid state can be converted to ionized gas using matrix-assisted laser-desorption ionization (MALDI). MALDI uses a coherent laser to excite, vaporize, and ionize the solid phase sample from a crystalline matrix. The efficiency requirements, number, distribution, and type of created ion will vary depending on ionization method.1
Mass separation
Once ionized, gas-phase ions under vacuum are separated by mass and charge. Generally, mass spectrometers are categorized by the mass analyzer component. Quadrupole mass spectrometers are among the most commonly used in the clinical laboratory, and use four electrodes to scan voltage ranges, creating unique ion trajectories specific to a single m/z range. Typically, quadrupole MS systems are highly quantitative, simple to use, and have relatively high throughput.1 In contrast, ion trap spectrometers have a limited capacity for containing large numbers of ions; thus their dynamic range of quantification is relatively narrow. This is particularly troublesome when attempting to simultaneously analyze multiple ions occurring over different or wide dynamic mass ranges.4 Ion trap mass spectrometers have high throughput and are relatively low cost and versatile. Time-of-flight (TOF) MS is among the fastest growing mass spectrometer available for use in biomedical and clinical research. Advantages of TOF-MS are a nearly unlimited m/z range, medium to high resolution, high resolution over a full mass spectrum, and very high m/z accuracy. Weaknesses include a limited dynamic range due to detector saturation during high ion currents.
Fourier transfer-ion cyclotron resonance (FTICR) spectrometers are currently rare in the clinical laboratory, though applications in drug screening have been documented.5 For FTICR, separation of ions is accomplished based on rotation, under a magnetic field, depending on their m/z. Compared to ion trap mass spectrometers, FTICRs are very similarly versatile but exhibit better resolution and mass accuracy.
Tandem MS (MS/MS) combines two or more mass analysis techniques in series. Commonly, the first mass analyzer is used to isolate the target m/z value, also known as the precursor ion. Between the first and second mass analyzer, the precursor ions are fragmented into product ions. The second mass analyzer allows the passage of a range of ions of different m/z values to reach the detector, either continuously or stepwise. Many commercially available systems use some form of tandem MS, and for good reason. Tandem MS significantly reduces the likelihood of interferences, and is highly selective and quantitative.1
Signal detection and analysis
Once filtered by the mass analyzer, ions bombard the ion counting or A-to-D conversion detection element. The detector gives an electronic response proportional to the number of targeted ions present. This electronic signal is then interpreted by a computer with bioinformatic software, which controls various instrument parameters and processes the data.
Mass spectrometer selection
The relative strengths and weaknesses associated with different mass separation configurations make choosing the most appropriate mass spectrometer challenging. In selecting the appropriate mass spectrometer for research or clinical needs, the following considerations should be explored: throughput (instrument robustness), versatility (variation of assay or sample type), analytes of interest (e.g., small, polar or volatile metabolites), qualitative (screening) or quantitative (diagnostic) requirements, price, and technical support (response time).
Clinical utility
Proteomics is the study of proteins, how they are modified, when and where they are expressed, how they are involved in metabolic pathways, and how they interact with one another.6 The holy grail of clinical proteomics is to identify a biomarker for a disease such as cancer. Early detection, which permits evidence-based treatment during early stages of disease, provides the greatest efficacy.7 Beyond diagnosis, mass spectrometry shows promise in preventative medicine, identifying patients at risk for developing diseases, or monitoring patients during treatment and thus enhancing the physician’s ability to give accurate prognosis.
Cancer biomarkers
With more and better therapeutic options and earlier diagnosis, more people are dying with cancer than from cancer in developed countries.8 Cancer biomarkers are becoming increasingly valuable tools for the prediction, diagnosis, prognosis, and long-term management of the disease. Mass spectroscopy, specifically MALDI imaging mass spectrometry, allows clinicians to simultaneously analyze multiple proteins and peptides along with their relative spatial distribution throughout the tissue of interest.
Sepsis
Sepsis affects more than 750,000 patients in the U.S., and is a leading cause of death worldwide.9,10 Culturing techniques are labor intensive, time consuming, require bacteriological expertise, and have been shown to be inaccurate when trying to identify fastidious organisms or those exposed to antibiotics.11 As an adjunct to blood culture, growth-independent methods of infectious disease testing may dramatically decrease the therapeutic turnaround time from suspected sepsis to adequate, targeted, antimicrobial treatment. For example, matrix-assisted laser desorption ionization time-of-flight mass spectroscopy (MALDI-TOF MS) can directly identify pathogens from positive blood culture samples in approximately 45 min.12
Heart disease
Detailed lists of identified cardiovascular biomarkers have been published however, few are routinely used in diagnosis of heart disease.13,14 Nevertheless, cardiovascular biomarkers show promise in determining myocardial mechanisms associated with immune response following heart transplant. Moreover, left ventricular assist devices may circumvent the need for heart transplant, and proteomic techniques utilizing mass spectrometry may provide a suitable diagnostic and monitoring tool for identifying opportunities for use of this less intrusive and often more practical option.15
Point-of-care mass spectrometry
Future visions of mass spectrometry include further automation, miniaturization, and ultimately point-of-care applications in native sample testing for diagnosis and therapeutic drug monitoring. Target analytes likely to be addressed by MS in a point-of-care format include disease-specific biomarkers and drug or drug metabolites, including anesthetics.16 Ion-trap mass analyzers offer compelling compromises between performance and ease of miniaturization. Novel atmospheric pressure interfaces and ionization methods allow for direct analysis of complex samples with minimal preanalytical processing.17,18 Field-portable MS units are commercially available from Griffin Analytical Technologies (West Lafayette, IN), Torion Technologies Inc. (American Fork, UT), and Bruker Daltonics Inc. (Billerica, MA). Currently, applications of miniature mass spectrometry focus primarily on environmental and biodefense testing.
Conclusion
Proteomics, facilitated in part by mass spectrometry, represents a fundamental bridge between evidence-based medicine and personalized medicine. A bedside or near-patient critical care plus therapeutic drug monitoring device would allow for rapid and early diagnosis, followed by patient specific therapies and real-time therapeutic drug monitoring.
Please check out our Mass Spectrometers section for more information or to find manufacturers that sell these products.
References
-
CLSI. Mass Spectrometry in the Clinical Laboratory: General Principles and Guidance; Approved Guideline. CLSI document C50-A. Wayne, PA: Clinical and Laboratory Standards Institute; 2007.
- Neilson, K.A.; Naveid, A.A. et al. Less label, more free: approaches in label-free quantitative mass spectrometry. Proteomics 2011, 11, 535–53.
- Podwojski, K.; Eisenacher, M. et al. Peek a peak: a glance at statistics for quantitative label-free proteomics. Expert. Rev. Proteomics 2010, 7, 249–61.
- Hill, L. How a quadrupole mass filter works. Available at: http://www.jic.ac.uk/services/metabolomics/topics/lcms/single1.htm; accessed July 18, 2011.
- Ojanpera, L.; Pelander, A. et al. Application of accurate mass measurement to urine drug screening. J. Anal. Toxicol. 2005, 29, 34–40.
- Tyers, M.; Mann, M. From genomics to proteomics. Nature 2003, 422, 193–7.
- Schilsky, R.L. Personalized medicine in oncology: the future is now. Nat. Rev. Drug Discov. 2010, 9, 363–9.
- Maisel, A.S.; Bhalla, V. et al. Cardiac biomarkers: a contemporary status report. Nat. Clin. Pract. Cardiovasc. Med. 2006, 3, 24–34.
- Dellinger, R.P.; Levy, M.M. et al. Surviving sepsis campaign; international guidelines for management of severe sepsis and septic shock: 2008. Crit. Care Med. 2008, 36, 296–327.
- Angus, D.C.; Linde-Zwirble, W.T. et al. Epidemiology of severe sepsis in the United States: analysis of incidence, outcome, and associated costs of care. Crit. Care Med. 2001, 29, 1303–10.
- Flayhart, D.; Borek, A.P. et al. Comparison of BACTEC PLUS blood culture media to BacT/Alert FA blood culture media for detection of bacterial pathogens in samples containing therapeutic levels of antibiotics. J. Clin. Microbiol. 2007, 45, 816–21.
- Leggieri N.; Rida, A. et al. Molecular diagnosis of bloodstream infection: planning to physically reach the bedside. Curr. Opin. Infect. Dis 2010, 23, 311–19.
- Vasan, R.S. Biomarkers of cardiovascular disease: molecular basis and practical considerations. Circulation 2006, 113, 2335–62.
- Matt, P.; Carrel T. et al. Proteomics in cardiovascular surgery. J. Thorac. Cardiovasc. Surg. 2007, 133, 210–14.
- Maisel, A.S.; Bhalla, V. et al. Cardiac biomarkers: a contemporary status report. Nat. Clin. Pract. Cardiovasc. Med. 2006, 3, 24–34.
- Kissinger, P.T.; Laughlin, B.C. et al. Developing a point-of-care mass spectrometer. IVD Technology 2010, 18, 23.
- Xu, W.; Manicke, N. et al. Miniaturization of mass spectrometry analysis systems. JALA 2010, 15, 433-–9.
- Zhu, Z.; Xiong, C. et al. Characterization of bioparticles using a miniature cylindrical ion trap mass spectrometer operated at rough vacuum. Analyst 2011, 136, 1305–9.
Keith Brock, BS is with UC Davis-LLNL Point-of-Care Technologies Center (NIBIB, NIH), Point-of-Care Testing Center for Teaching and Research (POCT•CTR), Pathology and Laboratory Medicine, School of Medicine, University of California, Davis, CA 95616, U.S.A.; tel.: 951-231-5581; e-mail [email protected].