Featured Article
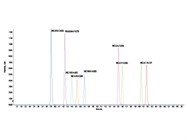
The Safe Drinking Water Act (SDWA) is one of multiple environmental and public health and safety-driven pieces of U.S. legislature to come out of the environmentalism of the 1970s. Prior to the SDWA, few regulations existed to define, measure, or limit potentially hazardous contamination in drinking water, and of these many were defined under state or local control. The SDWA passed in 1974, securing the U.S. EPA as the key responsible agency for monitoring and enforcing the public’s drinking water safety from contamination.
UCMR4 and microcystins
The Unregulated Contaminant Monitoring Rules (UCMR) is the result of the 1996 SDWA amendment requirement that the U.S. EPA continually seek to identify and monitor potentially harmful or relevant contaminants that may be currently unregulated. It is the first such national drinking water contaminant occurrence data-collection program. Monitoring certain water systems of varying size serviced populations to test for new contaminants, which do not yet have drinking water limits or other occurrence data, is intended to inform the agency as to which potential pollutants necessitate the creation and enforcement of maximum contaminant levels (MCLs) or other regulatory requirements. This list of unregulated chemicals is revisited and reprioritized every five years using data collected and reported by water treatment authorities and water providers.
In 2012, the third list of contaminants (UCMR3) replaced the previous list (UCMR2). Beginning in 2018, the fourth version of these (UCMR4) will go into effect with the latest suite of target contaminants defined for monitoring by water services across the U.S. This fourth version of the UCMR list has among its defined unregulated contaminants a suite of microcystins, a unique compound class of naturally occurring toxic products of aquatic microfaunal communities (algae, cyanobacteria). Water systems that use surface water and/or groundwater under the direct influence of surface water as their source will monitor for microcystins during a consecutive four-month period from March 2018 through November 2020. These and related cyanotoxins have been identified as human carcinogens. U.S. EPA 10-day drinking water health advisory for microcystins is 0.3 μg/L for infants and children up to six years old, and 1.6 μg/L for adults. World Health Organization (WHO) MC-LR provisional guideline is 1 μg/L. Minimum reporting levels (MRLs) in the UCMR4 list range from 0.005 to 0.09 μg/L based on EPA standard Method 544.
LC-MS/MS analysis
Organic contaminants in drinking water sources and treated streams, whether anthropogenic or endogenous, are commonly analyzed using LC-MS/MS. EPA Method 544 utilizes LC-MS/MS with up-front concentration of water samples using solid-phase extraction (SPE). Triple-quadrupole LC-MS/MS systems are generally considered the gold standard for these and similar applications due to the sensitivity and robustness of the workflow, as well as the ability to analyze liquid phase samples such as drinking water with minimal sample preparation. Multiple reaction monitoring (MRM) data acquisition using triple-quadrupole technology relies on the monitoring of a compound’s transition from a precursor ion to a corresponding fragment ion. This isolation of the target transition from constituents of other masses and other precursors to fragment transitions is what imparts the desired selectivity and specificity of these methods. MRM workflows are known for being highly sensitive and having a linear response range over several orders of magnitude of signal response, or sample concentration. These characteristics have for decades made LC-MS/MS workflows with MRM data acquisition a robust and widely adopted option for laboratories performing drinking water analysis of low-level organic contaminants.
The problem with false positives
Environmental testing labs utilizing triple-quadrupole mass spectrometry or other techniques to detect, identify, and quantify microcystins and other organic water contaminants are frequently confronted with the need for highly reliable confirmatory information when it comes to analyte identification in real-world samples. However, such complex environmental samples can be analytically challenging in their often unpredictable and highly variable background constituents, which may produce interfering or coeluting matrix peaks. Because of the severe human health concerns around toxic or carcinogenic contaminant compounds such as microcystins, it is crucial to be able to confirm any positive hits in water sources intended for use in communities by people. To this end, there has been increased interest and adoption of high-resolution/accurate mass (HRAM) mass spectrometer systems. Advantages gained include the ability to garner more specificity and qualitative confirmation of compound identifi cation, while maintaining the capacity for sensitive and robust routine quantitation. Methods and data are presented that demonstrate use of HRAM for accurate and sensitive quantitation of microcystins and nodularin in water samples.
Experimental
Chemical standards obtained from Enzo Life Sciences (Farmingdale, NY) were used to create a mixed stock by diluting standards in methanol to yield 500 μg/L for MC-RR and Nodularin-R, and 2000 μg/L for MC-LA, MC-LF, MC-LR, MC-LY, MC-LW, MC-YR, and MC-WR. Chromatographic separation was achieved under gradient conditions using a Kinetex C8 column (2.6 μm particle size, 100 × 3 mm) (Phenomenex, Torrance, CA) and flow rate of 0.500 mL/min. The mobile phases were water with 0.1% formic acid (A) and acetonitrile with 0.1% formic acid (B). Column oven temperature was 40 °C and a 20-μL injection was used. Run time was 11 minutes for the full gradient. An example elution profile is shown in Figure 1.
Figure 1 – Chromatographic separation of eight microcystins and one nodularin. Separation was achieved using a SCIEX ExionLC AD system and a Kinetex C8 column over an 11-minute gradient with water and acetonitrile.The SCIEX X500R QTOF system with the Turbo V source (SCIEX, Framingham, MA) was operated in positive mode electrospray ionization (ESI). The TOF (time of flight)-MS scan was conducted over a range of 500–1100 m/z. An MRMHR experiment included two transitions monitored for each analyte. For each transition in the acquisition method, the precursor ion was defined for the target analyte, and a mass range was defined that would encompass the expected fragment ion. Optimized instrument voltages were designated for each transition based on optimized values determined during method development. Additionally, chromatographic retention time (RT) values were specified for each MRMHR transition. Data acquisition and processing were performed using SCIEX OS software. Calibration curves were generated from the injection of seven concentration levels from 0.12 to 312 µg/L. Linear regression models with 1/× weighting were used to fit all calibration curves, and 1-point Gaussian smooth was applied to data for calibration and sensitivity evaluation. For the evaluation of method sensitivity, the limit of detection (LOD) was defined as the lowest calibrator with peak-to-peak signal to noise ≥3. The limit of quantitation (LOQ) was defined as the lowest calibrator with peak-to-peak signal to noise ≥10.
Quantitation of microcystins
Quantitation was achieved by utilizing the high-resolution TOF-MS data of each target compound. MRMHR transitions were acquired and used for quantitation and confirmation. Both the TOF-MS and MRMHR scans occur continually throughout the data acquisition of a single sample, and processing of the acquired data can utilize either or both. Comparison of these two types of analyses indicates that quantitation of the target microcystins using the TOF-MS extracted accurate mass of each is sensitive, linear, and precise; this approach demonstrates better performance compared to MRMHR for quantitation.
TOF-MS data was processed using known analyte charged monoisotopic masses (m/z) and a mass extraction width (XIC width) of 0.02 Da. Table 1 shows the extracted precursor masses used. Microcystins in the analyzed suite have MW >900. Ionization was performed in positive ion mode, but it is noted that the target MCs will protonate to form [M+H]+ species as well as doubly charged species. Eight of the nine analyzed targets show the singly charged species as dominant; it was therefore used for monitoring and quantitation. However, MC-RR showed the +2 charge as a greater intensity precursor than [M+H]+. The m/z values monitored for this precursor reflect the choice of the [M+2H]++ species for maximized sensitivity (Table 1). The 11-minute chromatographic gradient with the Kinetex C8 column achieved baseline separation for all compounds (Figure 1).
Table 1 – Accurate masses for microcystins and nodularin*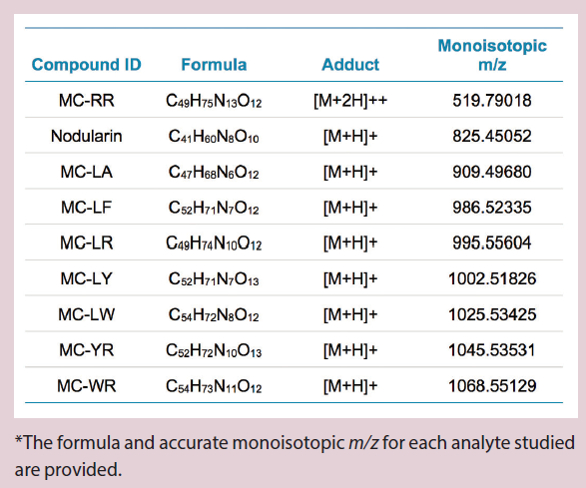
The LOD and LOQ concentrations for all compounds were demonstrated to be well below the lower range of the calibration curve (lowest calibrator 0.12 μg/L) when analyzed using TOF-MS data. Signal-to-noise ratios at 0.12 µg/L ranged from 27 (for MC-LR) to 91 (for MC-RR), demonstrating that lower concentrations may be detected and quantified using this method. Precision is shown as the %CV of triplicate analyses and is also listed in Table 2 for two concentration levels. Reproducibility is excellent, with all %CV values of 7% or less for the TOF-MS data. Linear dynamic range was also calculated and shown to be between 2.5 and 3.4 orders of magnitude. MRMHR data showed LOQ concentrations in the range of 1–3 μg/L. Precision at the low levels is also reduced compared to the TOF data, likely as a result of the decrease in the absolute signal available in the MS/MS data.
Table 2 – Quantitative analysis method performance*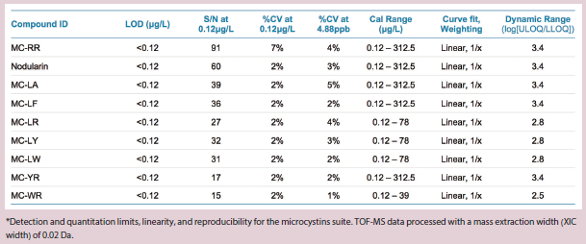
Use of HRAM data quality for confirmation
Figure 2 – Target identification points of confirmation. Identification and quantitation of microcystins in unknown samples can be achieved with high confidence using HRAM analysis due to multiple points of matching using accurate mass, MRMHR, isotope pattern matching, ion ratio, and chromatographic retention time.A key advantage of the HRAM approach to quantitation is the ability to apply multiple points of confirmation to avoid reporting false-positive detection (Figure 2). The five points of confirmation applied in this method are:
- Accurate mass of the target precursor
- Confirmation with the MRMHR transition and the accurate mass of the fragment ion
- Isotope ratio matching to theoretical pattern based on target formula
- Ion ratio matching between standard and unknown sample
- Retention time matching between standard and sample.
High-resolution TOF-MS analysis of microcystins shows mass resolution >40,000 across the range of precursor m/z. This mass resolution infers a high degree of specificity for the target analyte. Mass accuracy within 2 ppm reinforces confidence in the identification of the targets. High-resolution fragments collected using MRMHR also demonstrate excellent specificity and confidence from high mass resolution and mass accuracy. The TOF-MS pattern of resolved MS isotopes was compared to the theoretical isotope pattern for the empirical formulae of each microcystin; these data provide additional confirmation of the presence of the compound and are displayed in the results table within the SCIEX OS software. Precursor XIC and MRMHR transition data can also be grouped together in the data-processing module of SCIEX OS so that peak data corresponding to the same compound are processed as such; this allows the software to automatically evaluate ion ratios by comparing signal from multiple acquisition channels for a single analyte. Ion ratio comparison of sample data to that of standards provides another metric for qualitative matching and identity confirmation.
Summary
Quantitation of a suite of microcystins and nodularin was demonstrated using LC-MS/MS on the SCIEX X500R QTOF system. Utilizing the TOF-MS spectral data and extracting a narrow XIC for analyte precursor ions provided quantitation results with sensitive detection (<0.1 μg/L LOD) and high reproducibility (%CV<7%) at low concentrations. If used with the EPA Method 544 suggested concentration factor of 500-fold, these detection limits are theoretically extended to 0.0002 μg/L, attaining
the MRLs defined in the UCMR4 list.
Linearity was reported over 5–6 concentration levels and approximately three orders of magnitude with r-values from 0.95 to 0.99. MRMHR data also provided calibration and quantitation information, with sensitivity down to 1–3 μg/L. Concurrent MRMHR data allow for additional quantitative information and enhanced confidence in qualitative analysis without requiring additional injections or processing. Using the HRAM approach on the X500R system, confirmation of target identification can be attained using accurate precursor mass matching, isotope pattern matching, accurate fragment mass matching, ion ratio matching, and retention time matching. Verifying across these five points reinforces the ability to confirm the presence of the analyte and helps safeguard against reporting false positives.
Additional reading
- U.S. EPA. Office of Water (4304T). Statistical Design and Sample Selection for the Unregulated Contaminant Monitoring Regulation. Doc. no. 815-R-01-004; Aug 2001.
- U.S. EPA. Office of Water (4304T). Health and Ecological Criteria Division. Drinking Water Health for the Cyanobacterial Microcystin Toxins. Doc. no. 820R15100; June 2015.
- Health Canada. Federal-Provincial-Territorial Committee on Drinking Water. Cyanobacterial Toxins–Microcystin-LR; July 2002.
- World Health Organization. Cyanobacterial Toxins: Microcystin-LR in Drinking Water. Background document for preparation of WHO Guidelines for drinking-water quality. Geneva, 2003.
- Winslow, S.D.; Pepich, B.V. et al. Statistical procedures for determination and verification of minimum reporting levels for drinking water methods. Environ. Sci. Technol. 2006, 40, 281–8.
K.C. Hyland and Craig Butt are with SCIEX, 1201 Radio Rd., Redwood City, CA 94065, U.S.A.; tel.: 877-740-2129; e-mail: [email protected]; www.sciex.com. April Quinn-Paquet is with SCIEX, Framingham, MA, U.S.A.